Living organisms can be thought of as heat machines and metabolism as the inner workings of the machine. The metabolism converts energy from the combustion of food into useful work (mostly chemical work that makes new material for the living cell) so efficiency (useful work rather than wasted heat) can be maintained.
The human machine has two main metabolic fuels: carbohydrate and fat. Human metabolism also has two goals. First, we have to maintain significant energy production, the useful part of which is usually measured by the availability of biological intermediates that can drive life processes. Second, we must maintain more or less constant levels of blood glucose. Some tissues, particularly those that make up the brain and nervous system, require glucose as a fuel, so hypoglycemia (low blood sugar) represents a clear danger. High blood sugar (hyperglycemia) is also not good.
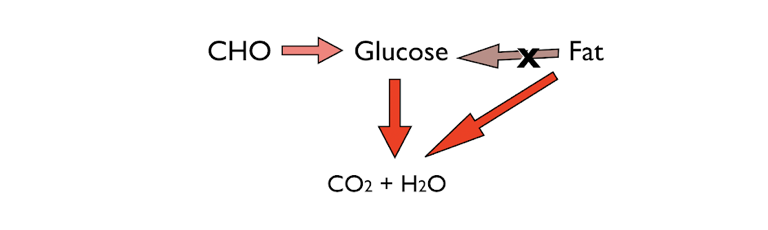
Figure 1: The two major fuels for energy: carbohydrate and fat. A critical characteristic of human metabolism is that, with a few exceptions, it cannot make glucose from fat.
To meet the goals of maintaining energy and keeping constant blood sugar, metabolism interconverts different compounds and fuels. This allows flexibility in what we eat and how we carry out other life processes. But there’s one problem: What happens when there’s no food? Our ancestors did not get three squares a day.
We all store enough fat to live for weeks or even months, so the first goal of maintaining energy is easily met. The threat in starvation, however, is failing to meet the requirement for constant blood glucose. Unlike fat storage, which can be extensive, we store very little glucose. The body’s rather limited solution to the need for storage is to form a polymer of glucose called glycogen. Glycogen is similar in structure to starch, the major dietary source of glucose beyond sugar itself.
Glycogen is stored predominantly in the liver and muscles. Under conditions of starvation, glucose units are chopped off the polymer (glycogen is highly branched and has many ends that can be used for rapid delivery of glucose). These units are exported from the liver and brought into circulation to supply the brain and other tissues. Glucose from muscle glycogen is not exported but rather used by the muscle itself, which is consistent with our view of the liver as the command center for metabolism and muscle as a consumer. Total glycogen from all the different muscles adds up to about 400 g. Liver glycogen provides another 100 g or so. As an energy source, muscle and liver glycogen combined could last for a couple days. Carbohydrate and the need to keep blood glucose constant is the critical part of the response to starvation.
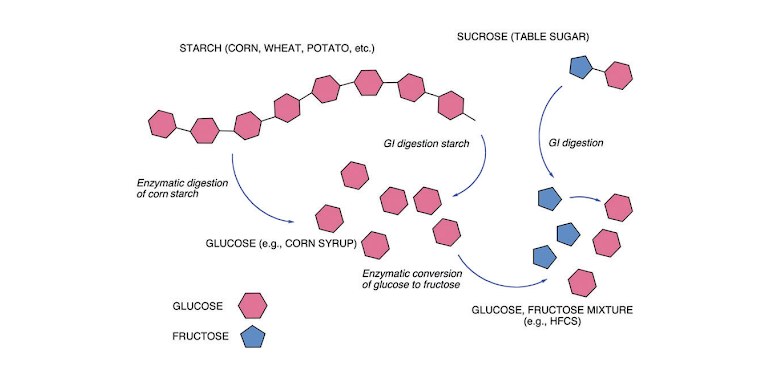
Figure 2: Summary of carbohydrate metabolism. The main dietary source of carbohydrate is the polymer starch. Digestion breaks starch down into individual glucose units that go into metabolism or are recycled into glycogen for storage. In addition to glucose, the other major sugar in the human diet is fructose. Fructose largely shows up in sucrose (table sugar: dimer of fructose and glucose) which is digested into the two separate sugars. (High-fructose corn syrup is a commercial product formed by turning some glucose into fructose).
Carbohydrate metabolism is summarized in Figure 2. Glucose is a six-carbon compound that folds into a hexagonal shape. Starch is a polysaccharide (polymer of glucose) and, depending on the source, may have hundreds or even a few thousand glucose units. Digestion leads to free glucose.
Gluconeogenesis
The liver is the command center for metabolism, sensing and providing for the needs of the tissues. It regulates blood glucose by sensing how much is out there and chopping off glucose units from stored glycogen. To replenish glycogen and maintain a continuing supply under conditions of starvation or overall low glucose levels, the liver carries out a process known as gluconeogenesis (GNG), which, as its name suggests, creates glucose from scratch. The starting material is other metabolites, mainly protein. Only a tiny fraction of all stored fat can be used for sugar production.
Control
How does the liver know when to switch on GNG? How do adipocytes (fat cells) know to rev up the breakdown of fat for fuel? There are two primary control agents, the first of which is the metabolites themselves. The liver responds to the levels of fat and sugar coming in. Second, the metabolic state is sensed and responded to by hormones, primarily insulin and glucagon.
Secreted by the beta cells of the pancreas in response to glucose, insulin is primarily anabolic (constructive); it stores fat and carbohydrate and increases protein synthesis. Control is frequently negative in that insulin suppresses the synthesis and activity of the second hormone, glucagon. Secreted by the alpha cells of the pancreas when glucose levels fall, glucagon stimulates the release of glucose from glycogen as well as the release of fatty acids from fat stores. Both activities are under the (negative) control of insulin.
We have global control through the hormones. If blood glucose is falling, glucagon is secreted, and glucagon stimulates the release of glucose from glycogen stores in the liver and breakdown of fat in adipocytes (fat cells). Insulin is a sign that times are good—maybe you just had dinner and it is time to store things. Insulin stops glucagon from adding more nutrients into the blood on top of what you just ate and stops gluconeogenesis from contributing glucose as well.
We now pretty much understand that the main effect of insulin, directly and indirectly, is to control hepatic production and the release of glucose. Textbooks traditionally emphasize that the reduction in blood glucose is due to insulin’s ability to increase uptake of glucose from the circulation, but we now consider this a secondary effect. This process becomes a problem when it stops working well. A person with Type 1 diabetes (formerly and sometimes still called insulin-dependent diabetes mellitus or IDDM) is characterized by a severe lack or absence of insulin. People with Type 1 diabetes cannot stop the release of glucose from the liver even when additional glucose is coming in from the diet, which leads to hyperglycemia, the most salient feature of diabetes. (People with Type 2 have sufficient insulin but poor response—they are said to be insulin-resistant—and hyperglycemia is primarily the failure to utilize glucose, which thus remains higher in the blood.)
Fat breakdown and storage
As in glucose metabolism, fat synthesis and breakdown is also under the control of insulin and glucagon. Figure 3 shows the E‑shaped structure of fats. The arms of the E are the fatty acids, and the backbone is the compound glycerol. The chemical name for fats is triacylglycerol (TAG), meaning three acids and one glycerol molecule (“acyl” is the adjective form of acid). Fat can be broken down into free fatty acids and glycerol; the process is called lipolysis (“lipo-” meaning “fat”; “-lysis” meaning “break down”). The fatty acids are largely hydrocarbon (long chains of carbon and hydrogen), exactly like gasoline and related hydrocarbon fuels. Chemically, they require essentially the same type of reaction (oxidation), but because we want it to happen at room temperature without a spark plug, we need to find a sophisticated mechanism. The storage of fat involves the formation of TAG from fatty acids and glycerol. Adipocytes maintain a lipolysis-synthesis cycle, regulated by insulin and glucagon in a manner similar to the synthesis and breakdown of glycogen. Insulin increases storage of TAG, and glucagon stimulates production of fatty acids from TAG.
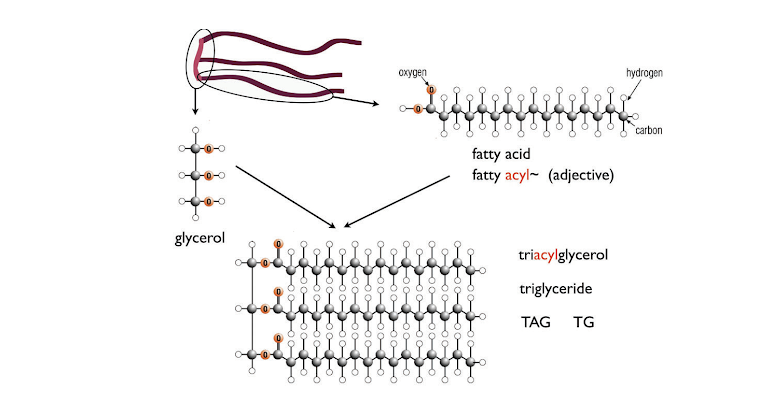
Figure 3: Fat (triacylglycerol; TAG) structure. Typical fatty acids have 20 or so carbon atoms. Fat cells continually break down and resynthesize TAG from fatty acids and glycerol.
Carbohydrate restriction and ketosis
The picture that is taking shape is of a process primarily regulated by carbohydrate. Glucose regulates the hormones, primarily insulin and glucagon. Fat plays a largely passive role in metabolism, as it is synthesized and broken down in response to carbohydrate. For weight loss, carbohydrate restriction is the strategy that follows from this idea.
Metabolically, it is the reduction in carbohydrate, not so much the reduction in total calories, that drives the response to starvation. The total calories, of course, will be part of the final product, but carbohydrate is the main controller. Carbohydrate, directly and through hormones, is said to be catalytic: In addition to providing fuel, it stimulates the disposition of other foods toward storage or oxidation. (The old literature used to say that fat was the powder and carbohydrate was the fuse.) A low-carbohydrate diet will avoid the problem of depleting body protein because of the protein that is included in the diet. The idea, then, is that the body follows the response appropriate to fasting but avoids the risk of losing lean body mass. Such diets were traditionally called “protein-sparing fasts,” or as George Cahill, a pioneer in physiology, put it, “the Atkins diet is a high-calorie starvation diet.” Practical low-carbohydrate diets tend to be high in fat (especially as compared to traditional recommendations) and are commonly abbreviated LCHF.
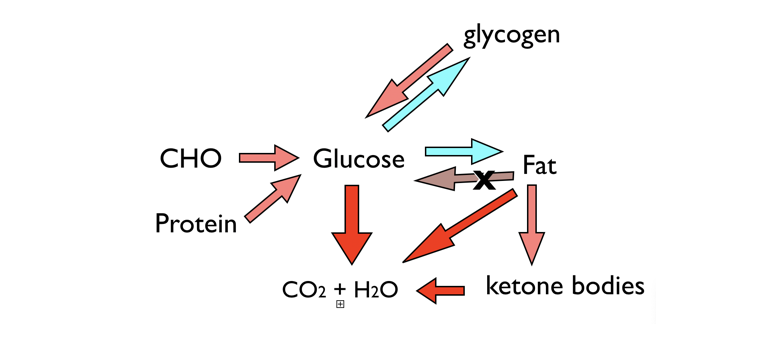
Figure 4: Metabolic function and ketosis. Two fuels (CHO and fat), supplied by dietary or stored macromolecules, are sources of energy from oxidation to carbon dioxide and water. A third fuel, ketone bodies, can be synthesized when glucose is low. Glucose can be stored in glycogen or converted to fat, but the key feature of metabolism is that you can’t convert fat to glucose. Protein can supply glucose, but in starvation, it will be body protein, and in this context, ketone bodies are “protein sparing” and can be oxidized as an alternative fuel, keeping the demand for GNG.
When there really is no food, evolution has selected for a mechanism for protein sparing by indirectly using fat. While the brain and central nervous system cannot use the fatty acids from fat, some of the oxidation of fat in the liver can be channeled into the creation of ketone bodies, which are small molecules that can be used by the brain and nervous system as well as other cells. Ketone bodies provide an alternative fuel to glucose and reduce the need for further utilization of protein for gluconeogenesis. The ketone bodies, beta-hydroxybutyrate (BHB) and acetoacetate, are four-carbon compounds that can reduce the need for glucose for many tissues. Under normal conditions, the brain requires about 130 g of glucose per day. After a few days in ketosis, this requirement will be cut in half. The signal for ketogenesis is the reduced carbohydrate, and the associated reduction in insulin and increase in glucagon. This means that inhibition of fat breakdown will be relieved as insulin falls and fatty acid is released into the circulation and transported to the liver. Very high fatty acid concentrations in the liver will lead to ketone body production, and at the same time, gluconeogenesis will be stimulated, resulting in ketone-body synthesis and gluconeogenesis.
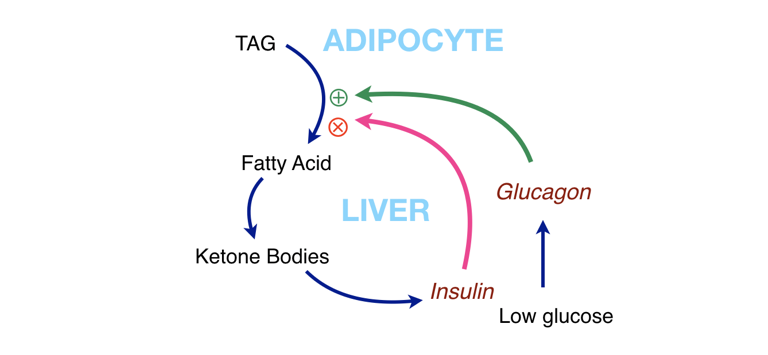
Figure 5: Interaction of metabolic components. Fat (TAG) can supply fatty acids for fuel, but this can also give us ketone bodies. To keep things from running away (too much fat breakdown, too many ketone bodies) ketone bodies stimulate insulin, which acts as negative feedback. The path of such a cycle is shown in Figure 6.
Ketone body regulation
Widespread interest in ketogenic diets is relatively recent, and their value even now may remain underappreciated. This is because we learned about ketone metabolism in only two contexts: in starvation, where ketone bodies are our friend, and in untreated Type 1 diabetes, where ketoacidosis becomes a real threat—a threat because the ketone bodies are acids, and acidosis (too much acid in the blood) can be life-threatening. Before the discovery of insulin, ketoacidosis could be a cause of death in people with Type 1 diabetes. The reason it is not a risk for nondiabetics on a ketogenic diet is that the ketone bodies themselves stimulate insulin release, which shuts down lipolysis, lowering fatty acids and turning off ketone body synthesis.
In other words, there is negative feedback. Under conditions of starvation or on a low-carbohydrate diet, the reduction in blood glucose is sensed by the pancreas and glucagon is secreted (and insulin is reduced). The breakdown in fat is stimulated by glucagon, and any inhibition by insulin is relieved as insulin levels go down. This leads to increased net production of fatty acids. The liver will now see an influx of fatty acids that can be used for the synthesis of ketone bodies. Thus, an alternative fuel for the brain is created in case it turns out that glucose really stays low. Now, if ketosis starts to get too high, the ketone bodies themselves will stimulate the pancreas to release insulin, which then will start to go back up. Glucagon will be inhibited, and together the two hormones will turn off lipolysis. The broad blue line in Figure 5 shows the cycle of stimulation and regulation of ketosis.
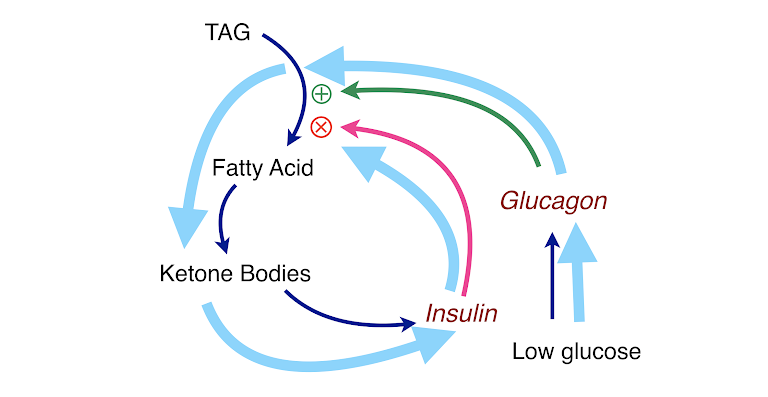
Figure 6: Keeping ketosis under control. Ketogenesis is regulated by a feedback loop (light blue arrows in the figure): low glucose ➛ insulin falls ➛ lipolysis goes up ➛ high fatty acids ➛ ketone bodies ➛ insulin increases ➛ lipolysis is inhibited ➛ lower fatty acids ➛ ketogenesis shuts off ➛ cells use glucose ➛ glucose falls ➛ insulin falls, etc. The bottom line is that a ketogenic diet does not have a risk of ketoacidosis.
Figures 5 and 6 show how this system works, but it is important to understand that this process is not like a pinball machine. In reality, it all happens at once: TAG is constantly being made and broken down, and glucose and hormones are sensed on the surface of cells. It is all in what is called a steady state—changes are accommodated by feedback, and biology tries to keep it all stable. That is why one Snickers bar may not “kick you out of ketosis” and why you have to do something drastic to lose weight.
Conclusion
Living organisms exist in a steady state in which they generate energy by burning food and have evolved mechanisms to maintain more or less constant glucose (see figure below). When there is no food, many organisms can switch to a new state in which they use stored fat as fuel, provide constant glucose by gluconeogenesis (predominantly from protein), and provide a protein-sparing alternative fuel in ketone bodies. The main control of the steady state rests with carbohydrate (and indirectly with insulin and other hormones), and this can be used for health advantages by obtaining the benefits of fasting while sparing protein.
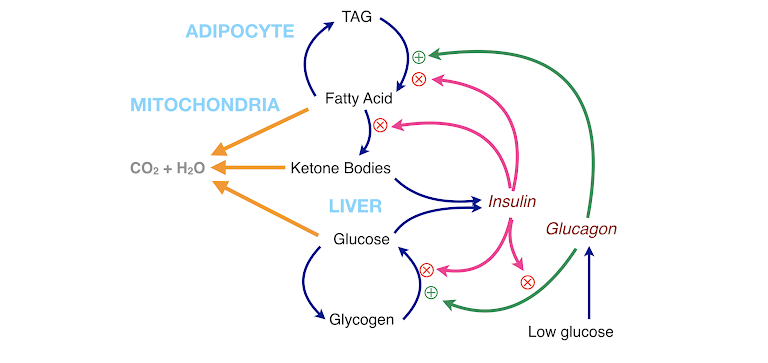
Figure 7: Summary of metabolism. The two fuels from the diet, carbohydrate and fat, and the ketone bodies that appear in response to carbohydrate restriction can be oxidized for energy (orange arrows). The fatty acid-TAG cycle and the glucose-glycogen cycle are regulated by insulin (which inhibits breakdown and favors storage) and by glucagon (which favors breakdown). Insulin itself regulates glucagon.
Richard David Feinman, Ph.D., is a professor of cell biology at the State University of New York Downstate Medical Center in Brooklyn, where he has been a pioneer in incorporating nutrition into the biochemistry curriculum. A graduate of the University of Rochester and the University of Oregon, Dr. Feinman has published numerous scientific and popular papers. He is the founder and former co-editor-in-chief (2004–2009) of the journal Nutrition & Metabolism. He is currently researching the application of ketogenic diets to cancer.
Comments on An Introduction to Metabolism
Very simple explanation of the Keto diet and how it works. We often only think about glucose as the main source of energy. Athletes should be proficient at using Carbs but also Fats to create energy! Especially now we knew the bad effects of functioning too much on carbs and it's effect on Insulin resistance and hyperinsulinemia.
Thank you! Very informative article and sure hope the masses are reading this!
An Introduction to Metabolism
2